.
## Who is Dr. David Stark?
Dr. David Stark worked with my physics professor, Dr. Chinmoy Bhattacharjee, back when they were both in graduate school at the University of Texas †at Austin. During their time at UT Austin, Dr. Stark worked on laser-plasma interactions, while my professor worked on plasma astrophysics. Stark has worked on various areas of physics from astronomy, quantum plasma, laser-plasma interactions, and has worked with my professor on general relativistic plasmas near compact objects.
Dr. Stark is currently a research scientist at Los Alamos National Laboratory, New Mexico. He has built his research career around the study of plasmas in a host of exotic systems. Early in his education, he modeled high-energy astrophysical phenomena, e.g., AGN jets, the Eta Carinae eruption, and this helped push him to do his graduate work in plasma physics. As a graduate fellow for the Department of Energy at UT Austin, he focused his dissertation on high-intensity laser-plasma interactions, focusing on relativistic effects that could be exploited for optical applications and radiation generation. After graduation, he held a post-doctorate at Los Alamos National Laboratory where he studied laser-ion acceleration before taking a permanent position there. He continues his work on laser-plasma interactions, mostly focusing on instabilities that can affect inertial confinement fusion (ICF) systems.
## Background & Motivation
About 60 years ago, laser technology really took off, which enabled the physics community to work with previously inaccessible physics and create new applications in technology.
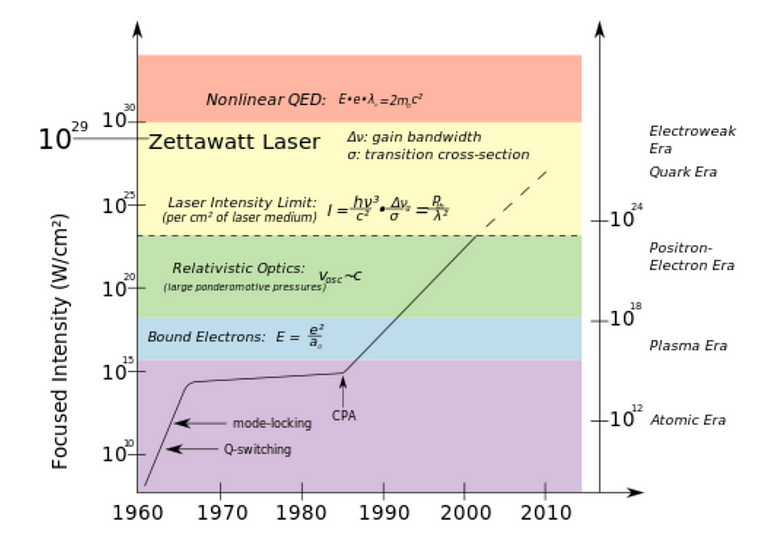
The following graph spans multiple decades and describes how intense can we get a laser, in Watts per square centimeter. In particular, we see how the development of Chirped Pulse Amplification (CPA) in 1985 allowed for really rapid growth.
We are able to go through several different regimes, even getting it to an intensity of 10^(20), which is the point where electrons can accelerate close to the speed of light using lasers. The progression estimate may seem just a little optimistic, but we are currently compatible of producing lasers with a focused intensity of between 10^(22) to 10^(23), and once we get above this we can start seeing 3D effects and all sorts of quantum effects.
This is still an exciting projection of what could happen in laser physics. Specifically, they’re using it to study laser-plasma interactions, where the lasers are ionizing targets very quickly and creating plasmas. Then suddenly there are these charged particles in very strong electromagnetic fields, which creates a very complicated problem. However, it can be used to create radiation sources, or redistribute the energy possibly even for pair-production one day.
### Applications
Some of the applications that come up by studying the fundamental physics of these interactions:
* Laboratory Astrophysics
* Ion fast ignition: A lot of work at Los Alamos is focused on inertial confinement fusion, which uses lasers to basically, compress plasma pullets with the goal of nuclear fusion
* Ion Beam Applications: Hadron therapy, which has some medical purposes
* X-ray and Gamma-ray production: Generated by the super relativistic electrons that are generated by these interactions
### Simulations & Theoretical Using Particle-Cell Tools
Since this is a very complicated E&M problem essentially, the physics community has widely adopted these particle-in-cell codes, which are the primary workhorse that is used to do the simulations and experimental modeling. They’re kinetic codes, so they actually have and follow millions and billions of macro-particles on a spatial domain, and each macro-particle corresponds to a certain number of real particles. And with the more macro-particles you have the more realistic the simulation is, the better you can sample a distribution function and so forth.
### Relativistic Transparency
Light propagation through a plasma is usually constrained by a dispersion relation, which is a relationship between the frequency and the wave vector. And so there’s a critical threshold for only a given density target of n_critical or below. Meaning only certain frequencies can propagate through that target, and if it’s a high enough frequency then it can penetrate through, but if it’s a low enough frequency then it’s reflected.
For instance, in a laser-plasma interaction where they’re heating a lot of these targets, the initial part of the pulse would come and hit the target and then it’s reflected because it’s over dense, however, as the target heats up and expands, eventually the target becomes transparent and the rear of the laser pulse is transmitted through, and so we see that it becomes under-dense.
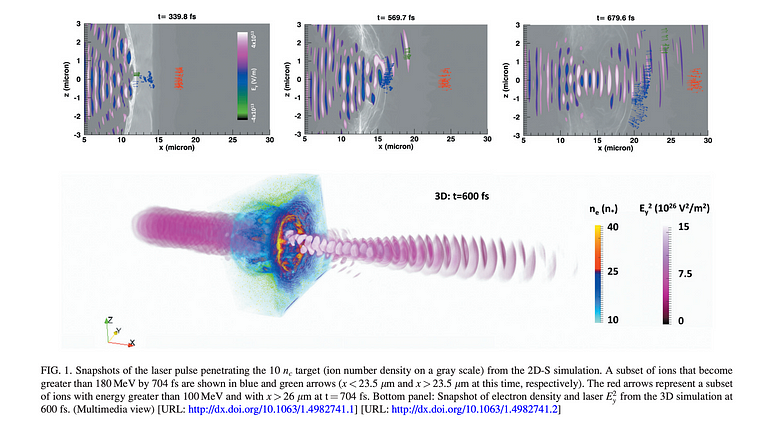
Now what’s actually happening in a relativistic laser-plasma interaction, is that as the particle becomes relativistically charged, as they get closer to the speed of light, their Lorentz factor, gamma, is going to be greater and greater than 1.
And so the plasma frequency is artificially reduced by that gamma factor. That means that the critical frequency becomes smaller. Frequencies that were initially reflected, now can become transparent. So this can become really appreciable for the critical frequency rejection in a very highly relativistic system. This is a phenomenon of relativistic transparency and it’s just essentially known as a relativistic mass weighting dropping the critical frequency.
This description is solely based on a relativistic mass, which means that it shouldn’t matter how the energy is distributed in the plasma.
So they were aiming to answer the question: What happens if there is relativistic thermal anisotropy in the system?
Basically, what if it’s hotter in the perpendicular direction, rather than the parallel direction?
As a proof of principle, they took a circularly polarized laser pulse and incidence it on a plasma slab. The plasma slab was then pre-initialized with a relativistic distribution function with anisotropy built into the distribution function.
The simulation resulted in projections along the bottom, with two linear polarizations. So the circularly polarized pulse has two linear components. And we can see that what is actually transmitted through the back of the target is actually only one of the polarizations on the bottom. Most of the pulse is reflected because we are in the intermediate regime of criticality. But we also see that we can get a preferential polarization of a circularly polarized pulse turning into the linearly polarized pulse after interacting with the target.
This suggests that the description of relativistic mass weighting can’t be the whole story because that would predict the exact same critical threshold for all the different polarizations. This suggests that there is a critical threshold that is polarization dependent in the plasma.
They then adopted, in featured simulations, a modified relativistic Maxwellian distribution function. However, they incorporated an anisotropy factor through epsilon. So when epsilon is zero, it’s a perfect standard relativistic Maxwellian distribution function, but it is tweaked by that parameter to understand what happens when there’s thermal anisotropy.
They are able to obtain the linear kinetic analysis of the distribution of the dispersion relations and then calculate the critical frequencies.
Each critical frequency shares the same isotropic factor, which is from the standard relativistic mass weighting, but they differ due to one of them being reduced by a lot more.
And so there is this anisotropy correction factor which is different based on the polarization. This tells us that the critical frequency is different between the two polarizations and in the y-polarized case, where it is lower, this means there is greater transparency that can be predicted analytically when there is a wave polarized along a hotter degree of freedom in a plasma.
They then inverted the polarization process and sent a linearly polarized pulse and because it will propagate differently with each polarization they were able to get a circularly polarized pulse out of the rear side. This is because the different phase velocities of each polarization are being shifted.
Dr. Stark did the same thing with a non-relativistic system by making things a lot colder and keeping the exact same anisotropy factor, and results showed that it simply isn’t an anisotropy effect but it is also relativistic.
This is potentially a new way that diagnostics can be used in laser-plasma interactions to characterize the state of the plasma and also use the plasma itself for potential new optical devices.
This is some of the work Dr. Stark did during his dissertation.
### Laser Ion Acceleration
How do ions accelerate when you have a high-intensity laser incident on a target? Especially when the target becomes transparent to the laser.
#### Background
When you try and transfer all this laser energy eventually there’s an ion population, and so electrons are used as an intermediary. One of the most common mechanisms that people look at is called Target Normal Sheath Acceleration (TNSA), which has a laser incident on a plasma target, which is over-dense. It’s not transparent, it’s just hitting the front of the target. The laser is heating up the electron at the front of the target, which is then accelerated through to the rear of the target where they set up this very strong virtual cathode, and so there’s a really strong field caused by these charged electrons at the rear of the target, and those then accelerate the ions off the back layer.
This is one of the most understood mechanisms of laser ion acceleration; it always accelerates normal to the target. However, in addition to this, there are many other ion acceleration mechanisms that have been discussed and discovered, but there’s not a lot of agreement on which acceleration mechanisms dominate, under which conditions, what type of energies you can achieve, and so during his post-doc Dr. Stark looked at ion acceleration mechanisms in particular, especially when under an accelerated regime, when the laser is allowed to penetrate the target.
They ran a 3D simulation of laser penetrating the target. There’s large scale reflection initially, but then it’s heating the plasma allowing it to expand, and also driving it to be relativistic so that eventually it becomes transparent.
In the particle-in-cell simulations, they tagged a lot of the particles. So they have the laser pulse coming in and it’s incident upon the target, but they tagged specific ions to monitor their location and energy so they can understand their dynamics.
#### Summary
Topics Discussed:
* Particle-In-Cell Codes
* Relativistic Transparency
* Thermal Anisotropy
* Laser Ion Acceleration
##### Sources
Dr. David Stark Bio + Image: Rutgers University — Newark Physics Department
D.J. Stark, L. Yin, B.J. Albright, and F. Guo, American Institute of Physics: Physics of Plasmas (2017).
“History of Laser Intensity.” Digital image. Wikipedia.org. https://en.wikipedia.org/wiki/Laser#/media/File:History_of_laser_intensity.svg
Physics Seminar On Relativistic Effects In High-Intensity Laser-Plasma Interactions
This seminar was presented by Dr. David Stark of Los Alamos National Lab and it touched upon some of the more unusual aspects of relativistic effects in laser-plasma systems. Who is Dr. David Stark?
